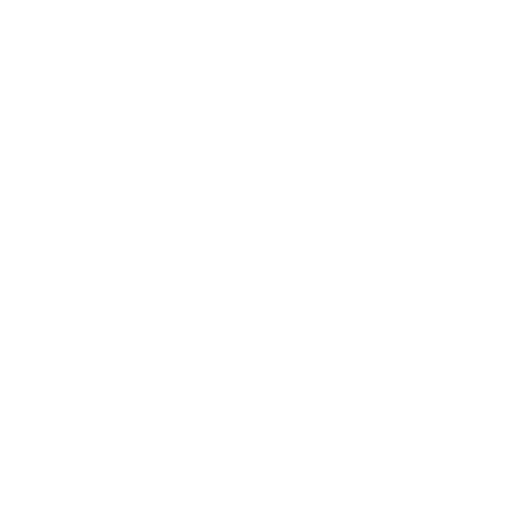

Introduction to Accuracy, Precision and Measurement Error
Some of the topics which have been explained are as follows:
Definitions of accuracy
Accuracy and Precision With Respect to Physics
Accuracy and Precision in the Context of Errors
Accuracy
Precision
Error
Uncertainty
Precision is a depiction of irregular errors, a proportion of statistical changeability.
Accuracy, However, Has Two Definitions
Usually, it is used to explain "it is a depiction of systematic errors, a proportion of statistical bias (on the researcher's or the experimenter's part); as these are causative of a distinction between an outcome and an "actual or true" value, ISO calls this trueness. On the other hand, ISO characterizes accuracy as portraying a blend of the two kinds of observational error above (irregular and methodical), so high accuracy requires both high precision and high certainty.
In the least difficult terms, given a lot of information focuses on repetitive measurements of a similar quantity or result, the set can be said to be exact if the values are near one another, while the set can be said to be accurate if their average is near the true value of the quantity being valued. In the first, common definition given above, the two ideas are autonomous of one another, so a specific arrangement of information can be said to be either accurate, or precise, or both, or neither of the two.
In the domains of science and engineering, the accuracy of an estimation framework is the degree of closeness of a quantity to that quantity’s actual or true value. The accuracy of an estimation framework, identified with reproducibility and repeatability, is the degree up to which repeated estimations under unaltered conditions demonstrate the equivalent results.
Although the two words accuracy and precision can be synonymous in everyday use, they are intentionally differentiated when used in the context of science and engineering. As mentioned earlier, an estimation framework can be accurate yet not precise, precise yet not accurate, neither or the other, or both. For instance, on the off chance that an investigation contains a systematic error, at that point expanding the sample size, for the most part, builds precision yet does not necessarily improve accuracy.
The outcome would be a consistent yet inaccurate series of results from the defective examination. Taking out the systematic error improves accuracy however does not change precision. An estimation framework is viewed as valid on the off chance that it is both accurate and precise. Related terms include bias (either non-arbitrary and coordinated impacts brought about by a factor or factors disconnected to the independent variable) and error (random fluctuation).
(Image will be uploaded soon)
Accuracy and Precision With Respect to Physics
Accuracy can be described as the measure of uncertainty in an experiment concerning an absolute standard. Accuracy particulars, more often than not, contain the impact of errors because of gain and counterbalance parameters. Offset errors can be given as a unit of estimation, for example, volts or ohms, and are free of the size of the input signal being estimated.
A model may be given as ±1.0 millivolt (mV) offset error, paying little mind to the range of gain settings. Interestingly, gain errors do rely upon the extent of the input signal and are communicated as a percentage of the reading, for example, ±0.1%. All out or total accuracy is hence equivalent to the aggregate of the two: ± (0.1% of information +1.0 mV).
Precision depicts the reproducibility of the estimation. For instance, measure a steady state's signal repeatedly. For this situation in the event that the values are near one another, at that point, it has a high level of precision or repeatability. The values don't need to be the true values simply assembled together. Take the average of the estimations and the difference that is between it and the true value is accuracy. If upon repeated trials the same values or nearest possible values are observed then that becomes precision.
Accuracy and precision are also predominantly affected by another factor called resolution in Physics.
Resolution May Be Expressed in Two Ways
It is the proportion between the highest magnitudes of signal that is measured to the smallest part that can be settled - ordinarily with an analog to-computerized (A/D) converter.
It is the level of change that can be hypothetically recognized, usually communicated as a certain number of bits. This relates the number of bits of resolution to the true voltage estimations.
So as to decide the goals of a framework regarding voltage, we need to make a couple of estimations. In the first place, assume an estimation framework equipped for making estimations over a ±10V territory (20V range) utilizing a 16-bits A/D converter. Next, decide the smallest conceivable addition we can distinguish at 16 bits. That is, 216 = 65,536, or 1 section (part) in 65,536, so 20V÷65536 = 305 microvolt (uV) per A/D tally. Along these lines, the smallest hypothetical change we can recognize is 305 uV.
However, unfortunately, different elements enter the condition to reduce the hypothetical number of bits that can 3-Jan-2022be utilized, for example, noise (basically any form of disruption that may cause an imbalance in an environment to disrupt the experiment). An information procurement framework determined to have a 16-bit goal may likewise contain 16 counts of noise. Thinking about this noise, the 16 counts only equals 4 bits (24 = 16); along these lines, the 16 bits of resolution determined for the estimation framework is reduced by four bits, so the A/D converter really resolves just 12 bits, not 16 bits.
A procedure called averaging can improve the goals, yet it brings down the speed. Averaging lessens the noise by the square root of the number of samples, consequently, it requires various readings to be included and afterward divided by the total number of tests. For instance, in a framework with three bits of noise, 23 = 8, that is, eight tallies of noise averaging 64 tests would lessen the noise commitment to one tally, √64 = 8: 8÷8 = 1. Be that as it may, this system can't decrease the effects of non-linearity, and the noise must have a Gaussian dispersion.
Sensitivity is an absolute amount, the smallest total measure of progress that can be identified by an estimation. Consider an estimation gadget that has a ±1.0 volt input extend and ±4 checks of noise if the A/D converter resolution is 212 the crest to-top affectability will be ±4 tallies x (2 ÷ 4096) or ±1.9mV p-p. This will direct how the sensor reacts. For instance, take a sensor that is evaluated for 1000 units with a yield voltage of 0-1 volts (V). This implies at 1 volt the equal estimation is 1000 units or 1mV equals one unit. However, the affectability is 1.9mV p-p so it will take two units before the input distinguishes a change.
Accuracy and Precision in the Context of Errors
When managing error analysis, it is a smart thought to recognize what we truly mean by error. To start with, we should discuss what error isn't. An error isn't a silly mistake, for example, neglecting to put the decimal point in a perfect spot, utilizing the wrong units, transposing numbers, etc. The error isn't your lab accomplice breaking your hardware. The error isn't even the distinction between your very own estimation and some commonly accepted value. (That is a disparity.)
Accepted values additionally have errors related to them; they are simply better estimations over what you will almost certainly make in a three-hour undergraduate material science lab. What we truly mean by error has to do with uncertainty in estimations. Not every person in the lab will have the same estimations that you do and yet (with some undeniable special cases because of errors) we may not give preference to one individual's outcomes over another's. Therefore, we have to group sorts of errors.
By and large, there are two sorts of errors: 1) systematic errors and 2) arbitrary or random errors. Systematic errors are errors that are steady and dependable of a similar sign and in this way may not be diminished by averaging over a lot of information. Instances of systematic errors would be time estimations by a clock that runs excessively quick or slow, removing estimations by an inaccurately stamped meter stick, current estimations by wrongly aligned ammeters, and so forth. Usually, systematic errors are difficult to relate to a solitary analysis. In situations where it is critical, systematic errors might be segregated by performing tests utilizing unique strategies and looking at results.
On the off chance that the methodology is genuinely unique, the systematic errors ought to likewise be unique and ideally effectively distinguished. An investigation that has few systematic errors is said to have a high level of accuracy. Random errors are an entire diverse sack. These errors are delivered by any of various unusual and obscure varieties in the examination.
Some of the examples may include changes in room temperature, changes in line voltage, mechanical vibrations, cosmic rays, and so forth. Trials with little random errors are said to have a high level of precision. Since arbitrary errors produce varieties both above and beneath some average value, we may, by and large, evaluate their importance utilizing statistical methods.
Accuracy
Accuracy alludes to the understanding between estimation and the genuine or right value. In the event that a clock strikes twelve when the sun is actually overhead, the clock is said to be accurate. The estimation of the clock (twelve) and the phenomena that it is intended to gauge (The sun situated at apex) are in the ascension. Accuracy can't be talked about meaningfully unless the genuine value is known or is understandable. (Note: The true value of measurement can never be precisely known.)
Precision
Precision alludes to the repeatability of estimation. It doesn't exactly require us to know the right or genuine value. In the event that every day for quite a while a clock peruses precisely 10:17 AM the point at which the sun is at the apex, this clock is exact. Since there are above thirty million seconds in a year this gadget is more precise than one part in one million! That is a fine clock in reality! You should observe here that we don't have to consider the complicated calculations of edges of time zones to choose this is a good clock. The genuine significance of early afternoon isn't critical in light of the fact that we just consider that the clock is giving an exact repeatable outcome.
Error
Error alludes to the contradiction between estimation and the genuine or accepted value. You might be shocked to find that error isn't that vital in the discourse of experimental outcomes.
Uncertainty
The uncertainty of an estimated value is an interim around that value to such an extent that any reiteration of the estimation will create another outcome that exists in this interim. This uncertainty interim is assigned by the experimenter following built-up principles that estimate the probable uncertainty in the outcome of the experiment.
FAQs on Accuracy, Precision and Error in Measurement
1. Are the Accuracy, Precision, and Error in Measurement articles available in PDF form?
Yes, the Accuracy, Precision, and Error in Measurement article are available in PDF form. Students can easily download the PDF on their devices for free. Keeping the PDF will be useful for students as they can refer to it even when there is no internet connection. They can use the PDF file anytime they want to without having to be online.
Thus, the Accuracy, Precision, and Error in Measurement PDF will be a very useful addition to their study routine.
2. How to download the Accuracy, Precision, and Error in Measurement PDF?
It is very easy for students to download the Accuracy, Precision, and Error in Measurement PDF. They can download the PDF by following these simple steps:
Search for “Accuracy, Precision, and Error in Measurement”
Click on the Download PDF button
A pop-up box appears, sign-up using your email or mobile.
If you already have an account, you can log in using your password.
A confirmation message will be sent on your device along with the Accuracy, Precision, and Error in Measurement PDF file.
The PDF file will get downloaded on your device too.
Thus, students can download the Accuracy, Precision, and Error in Measurement PDF in a very simple way.
3. Who prepared the Accuracy, Precision, and Error in Measurement PDF?
The Accuracy, Precision, and Error in Measurement PDF have been prepared by the expert faculty of Vedantu who have had a background in teaching this topic to students. They have a lot of knowledge about the challenges students face while studying the Accuracy, Precision, and Error in Measurement topics. The PDF has been prepared in such a manner that students can easily understand it while going through it.
Thus, downloading the Accuracy, Precision, and Error in Measurement PDF will be very advantageous for students in their studies.





